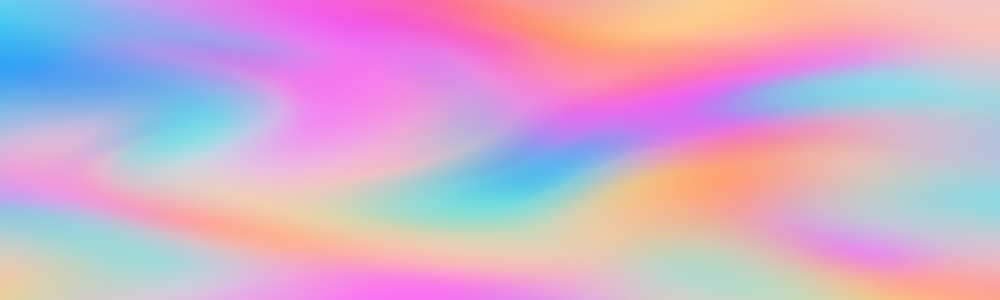
Rust lifetimes can feel like one of the trickiest parts of the language, especially when your code suddenly refuses to compile due to mysterious borrowing rules. This guide breaks lifetimes down into clear, practical sections to help you understand what they are, when they’re needed, and how to work with them confidently.
We’ll cover:
- What lifetimes are and why they exist
- Understanding borrowing and how the compiler uses lifetimes behind the scenes
- The basics of lifetime annotations
- Lifetimes in functions — including Rust’s elision rules
- Using lifetimes in structs and how to decouple them
- How lifetimes interact with generics
- Lifetimes in traits and trait bounds
- The
'static
lifetime and when to use it - How closures interact with lifetimes
Whether you’re just starting to write more complex Rust or you’ve been wrestling with lifetime-related errors, this guide is here to help you master the concept with real-world examples and insights.
Introduction
Rust’s ownership and borrowing system is one of its most powerful features, and also one of its most challenging for newcomers. By enforcing strict rules about how memory is accessed and shared, Rust ensures safety without relying on a garbage collector. Rust’s rules can prevent entire classes of bugs like use-after-free and data races.
But as programs grow more complex, especially when passing references between functions, storing them in structs, or combining them with generics, you’ll start encountering a new kind of concept: lifetimes.
Lifetimes are Rust’s way of ensuring that references remain valid only as long as the data they point to is valid. Lifetimes exist entirely at compile time, and they’re crucial for preventing dangling references, where something points to memory that no longer exists.
Lifetimes touch many areas of Rust, so we’ll break it up into two posts. In this post, we’ll get started looking at Rust lifetimes:
- What lifetimes are and how they relate to references
- When you need lifetimes and why you need to annotate them
- Lifetime syntax and behavior
- How to work with them in functions, structs, and more
This guide is written for developers who are at least somewhat comfortable with Rust’s basic ownership model and are starting to write code involving references that span across functions, structs, or trait implementations.
If you need a refresher on Rust ownership, borrowing, and references, this ByteMagma post might help:
Ownership, Moving, and Borrowing in Rust
What Are Lifetimes?
First of all, it’s important to understand that lifetimes
always relate to references
.
References are pointers to data — they are not the data itself, but rather the address where the data is stored in memory.
So, if you have a reference to some data — that is, the memory address of that data — and the data the reference points to becomes invalid (for example, goes out of scope), what happens to the reference?
Now the reference points to nothing valid. It becomes a dangling reference — a problem that causes serious bugs in languages like C and C++, such as undefined behavior, memory corruption, or crashes.
In Rust, we avoid these problems with lifetimes. Lifetimes are annotations
we add to our code that uses references, and these annotations act as promises we make to the compiler. We promise that the reference will be valid for at least as long as the data it points to.
At compile time, the compiler analyzes the entire codebase and emits an error if any lifetime promise is violated. If a function takes two references, and we use lifetime annotations in our function definition making a promise about how long those references will be valid, and we violate that promise in the scope that calls the function, the compiler will complain.
This is the beauty of lifetime annotations. The compiler ensures we write code that is memory safe, otherwise our code won’t compile.
Lifetime annotations don’t affect the runtime behavior or performance of your program, they exist purely for the compiler to verify that your references stay valid throughout their use.
Borrowing and Lifetimes
To understand lifetimes
you need to understand borrowing
. When you borrow a value in Rust using &T
(a shared, immutable reference) or &mut T
(a mutable reference), you’re creating a reference to the value for some period of time. That period of time, the scope during which the reference is valid, is the reference’s lifetime.
If we don’t use a reference, ownership of the value is moved rather than borrowed. When you move a value, the original value is no longer valid and usable. Usually that’s not what you want, which is why we use references.
Rust enforces the rule that the data being referenced must not be dropped while the reference is still in use. Additionally, when a value is borrowed as a shared (immutable) reference (&T
), it cannot be mutated for the duration of that borrow. When it’s borrowed as a mutable reference (&mut T
), it can be mutated, but only if no other references (shared or mutable) to the same data exist at the same time.
The Three Rules of References
Rust enforces a few core rules whenever you work with references:
- At any given time, you can have either:
- one mutable reference,
- OR any number of shared (immutable) references
- but not both — you cannot have a mutable reference while immutable references to the same data exist
- References must always be valid, so at any point in time, for a reference to be valid, the data the reference points to must be valid. You can’t use a reference to data that has been moved or dropped.
- The data referenced must live at least as long as the reference.
This is where lifetimes come into play, allowing the compiler to ensure references never outlive the data they point to.
These rules ensure memory safety and prevent race conditions at compile time. While they might seem strict at first, they form the foundation of Rust’s reliability—and lifetimes are the mechanism the compiler uses to enforce them behind the scenes.
These rules prevent common bugs like dangling pointers, use-after-free, and data races, all without needing a garbage collector.
The Rust borrow checker uses lifetimes behind the scenes to enforce these rules. In simple cases, it figures out everything for you. In more advanced situations, it may ask you to annotate lifetimes explicitly so that it can understand how your references relate.
If you need a refresher on Rust ownership, borrowing, and references, this ByteMagma post might help:
Ownership, Moving, and Borrowing in Rust
How the Compiler Uses Lifetimes
Unlike languages with garbage collectors (like Java or Go), Rust relies entirely on static analysis to ensure memory safety. That means the compiler must be certain that every reference is valid for its entire usage span before your code ever runs.
By requiring lifetimes to be consistent and properly scoped, Rust guarantees:
- No dangling references
- No use-after-free
- No race conditions caused by concurrent mutation and reading
This compile-time guarantee is one of Rust’s most valuable features, but it can also be one of the most frustrating when you’re first learning how lifetimes work. The key is to realize that lifetimes are not an additional burden; they are a precise tool that helps the compiler reason about your code the same way you do—just more thoroughly and more consistently.
Lifetime Annotations: The Basics
Before we start looking at the many ways lifetimes can appear and even be required in your code, let’s look at a simple example, just to get acquainted with basic lifetime annotation syntax.
fn first_word<'a>(msg: &'a str) -> &'a str {
match msg.find(' ') {
Some(pos) => &msg[..pos],
None => msg,
}
}
fn main() {
let message = "Frank is a premium user.";
let name = first_word(&message);
println!("Username: {}", name);
}
// Output:
// Username: Frank
This simple function takes a single string slice parameter (&str). It returns a string slice that can be one of two values:
- The first word of the passed in message if a space is found in the message
- The passed in message, if no space is found
Let’s break down the core pieces of lifetime annotation syntax in Rust:
first_word<'a>
This declares a named lifetime parameter called 'a
that can be used in the function signature. It indicates that references’ lifetimes will be tracked in this function.
msg: &'a str
We use the declared lifetime 'a
on the input parameter reference. This means that this input reference will be valid for the declared lifetime 'a
. It tells the compiler: “This reference must not outlive the data it points to.”
-> &'a str
We also use the declared lifetime 'a
on the function return type. This means the value returned from the function will be valid for the declared lifetime 'a
. It tells the compiler: “The returned reference must not outlive the data the input parameter points to”.
Note that similar to generics in which we typically use a capital T
for a single generic type parameter, or T and U
for two type parameters, or K and V
for the key and value of a generic HashMap, we typically use a lowercase letter 'a
for a single lifetime specifier, and 'a, 'b
for two, 'a, 'b, 'c
for three and so on.
But using 'a, 'b, 'c
, etc. for lifetimes and T, U, K, V
, etc. for generics is just the convention. You could use anything, even more that one letter, and that can lead to greater clarity on the purpose of code.
The calling scope — in this case, main()
— owns the data and passes a reference into the function. The lifetime annotations act as a contract, letting the compiler ensure that the input reference—and the returned reference—remain valid for the same span of time. The compiler checks this at the call site to ensure the promise is kept.
A lifetime annotation looks like a generic parameter, but it’s written with an apostrophe, like 'a
. This is similar to generics where we annotate a function with a generic type and use the generic type on the function parameters and return type:
// apply generic type T to parameters num1 and num2
// and to the return type
fn calculate<T>(num1: T, num2: T) -> T
We’re doing something similar with lifetime annotations:
// apply lifetime 'a to parameter msg
// and to the return type
fn first_word<'a>(msg: &'a str) -> &'a str
Note that in this example code, Rust actually doesn’t require explicit lifetimes—it could infer them. But writing them out here helps us understand lifetime annotation syntax and how lifetime tracking works.
Now that we’ve covered the theory, let’s move step by step through the key Rust features where lifetimes come into play — and see how to work with them in real code.
Lifetimes and Functions
When you pass references into a function, you’re telling Rust:
“I’m temporarily borrowing some data — don’t let it outlive its source.”
But the compiler needs to verify that this promise holds, especially when a function returns a reference. It must know:
How long is the input parameter reference valid? Where did it come from? Is it safe to use after the function ends?
That’s where lifetimes come in. Lifetime annotations don’t affect how your code runs — they just help the compiler track the scope and safety of references. In simple functions, Rust can often infer the lifetimes automatically. But when things get more complex — especially with multiple input references or returned references — we have to annotate lifetimes explicitly so the compiler can do its job and keep us safe.
This section covers:
- Elision rules to infer lifetimes
- Single input reference
- References in return types
- Two references with the same lifetime
- Two references with different lifetimes
If you need a refresher on Rust functions, this ByteMagma post might help:
Functions in Rust – The Building Blocks of Reusable Code
Let’s briefly revisit the example we used to present lifetime annotation syntax.
fn first_word(msg: &str) -> &str {
match msg.find(' ') {
Some(pos) => &msg[..pos],
None => msg,
}
}
This version of the function is identical except that we have removed the lifetime annotations. In this case lifetime annotations are unnecessary because the compiler is able to infer that the return value reference will have the same lifetime as the one input parameter reference.
How did Rust know the return value was safe? It used a built-in set of rules called lifetime elision — and in this case, they let us skip all the annotations.
Lifetime Elision: What the Compiler Can Infer Automatically
To make lifetimes less painful, Rust uses elision rules to infer lifetimes in simple cases. These rules cover many everyday patterns. Often you can consider a situation and refer to these lifetime elision rules to understand when you need lifetime annotations.
The three elision rules:
- Each reference parameter gets its own lifetime.
- If there’s exactly one input reference, the output gets that lifetime.
- If a method has a parameter
&self
or&mut self
, the output gets the same lifetime asself
.
In everyday English, “elision” means leaving something out—intentionally omitting something because it can be understood from context. In Rust, lifetime elision means the compiler fills in lifetime annotations for you when it can do so unambiguously, based on a set of built-in rules.
For our simple method first_word, the first and second elision rules apply. The one parameter gets a lifetime behind the scenes. There is exactly one input reference, so the return type gets that lifetime.
Because the input reference and the output (return) reference have the same lifetime, explicit lifetime annotations are unnecessary.
Two references with the same lifetime
Let’s get started with some code. Open a shell window (Terminal on Mac/Linux, Command Prompt or PowerShell on Windows). Then navigate to the directory where you store Rust packages
for this blog series, and run the following command:
cargo new lifetimes
Next, change into the newly created lifetimes
directory and open it in VS Code (or your favorite IDE).
Note: Using VS Code is highly recommended for following along with this blog series. Be sure to install the Rust Analyzer extension, it offers powerful features like code completion, inline type hints, and quick fixes.
Also, make sure you’re opening the lifetimes
directory itself in VS Code. If you open a parent folder instead, the Rust Analyzer extension might not work properly, or at all.
Note, as we work through the sections of this post, you can either comment out the current code with a multiline comment to keep it for future reference:
/*
CODE TO BE COMMENTED
OUT BEFORE ADDING NEW CODE
*/
Or you can just delete the current code if you don’t want to keep it for reference.
Our first example involved a single input reference with a single inferred lifetime. Now let’s look at an example of two input references with the same lifetime.
fn longer<'a>(arg1: &'a str, arg2: &'a str) -> &'a str {
if arg1.len() > arg2.len() {
arg1
} else {
arg2
}
}
fn main() {
let msg1 = String::from("Message Received!");
let msg2 = String::from("Your message was received!");
let longer_string = longer(&msg1, &msg2);
println!("Longer message: {}", longer_string);
}
// Output:
// Longer message: Your message was received!
This code defines a function longer() that takes two string slices (&str) and returns the longer of the two. In main() we create two string slice variables, pass them into the function, and prints out the return value.
If we remove the lifetime annotations from this function the compiler complains:
fn longer(arg1: &str, arg2: &str) -> &str {
if arg1.len() > arg2.len() {
arg1
} else {
arg2
}
}

This code fails to compile because it returns one of two input references — and Rust doesn’t know which one at compile time. Notice how the compiler error includes an example of how to introduce lifetime annotations to address the issue. You’ll want to closely examine lifetime error messages as they often include such suggestions.
In our code it is certain because we have defined two string slices of definite length, but what if this code is used later with user input? The compiler needs to know at compile time which lifetime to assign to the returned reference — and with two inputs, that’s ambiguous unless we annotate it.
Because there are multiple input references, Rust’s lifetime elision rules don’t apply — and the compiler asks us to be explicit.
We annotate the function with one lifetime <'a>
and we use that lifetime annotation on all three references (arg1
, arg2
, and the return value
). This tells the compiler:
“The reference we return must be valid for as long as both
arg1
andarg2
are valid.”
In other words, by using the same lifetime 'a
, we’re promising the compiler that the returned reference is valid for the full duration of both inputs — and the compiler enforces that by requiring 'a
to be no longer than the shorter of the two.
We’re not saying arg1
and arg2
actually have the same lifetime — just that the returned reference must be valid as long as both of them are.
To put this more simply, the return value cannot live longer than arg1, and the return value cannot live longer than arg2.
This is how Rust guarantees memory safety: it enforces this contract at the call site. If one of the references lives for less time than the other, and it’s the one that would be returned, the compiler won’t allow the function to be called.
Let’s change the code in main() to see how we can get a lifetime error even after specifying lifetime annotations.
fn longer<'a>(arg1: &'a str, arg2: &'a str) -> &'a str {
if arg1.len() > arg2.len() {
arg1
} else {
arg2
}
}
fn main() {
let longer_string;
let msg1 = String::from("Message Received!");
{
let msg2 = String::from("Your message was received!");
longer_string = longer(&msg1, &msg2);
}
println!("Longer message: {}", longer_string);
}

This error occurs because variable longer_string
lives longer than msg2
. Variable msg2 is defined in a code block but longer_string is defined outside the code block. When the code block ends variable msg2 goes out of scope and is dropped, but longer_string still has a reference to the msg2 data, which is no longer valid.
In this case the error message doesn’t have a suggested fix, so we need to examine the error message and consider what to change to address the issue.
Two possible fixes are to move the declaration of msg2 out of the code block, or move the println!() into the code block:
Move the declaration of msg2 out of the code block:
fn main() {
let longer_string;
let msg1 = String::from("Message Received!");
let msg2 = String::from("Your message was received!");
{
longer_string = longer(&msg1, &msg2);
}
println!("Longer message: {}", longer_string);
}
Move the println!() into the code block:
fn main() {
let longer_string;
let msg1 = String::from("Message Received!");
{
let msg2 = String::from("Your message was received!");
longer_string = longer(&msg1, &msg2);
println!("Longer message: {}", longer_string);
}
}
In this fix, even though longer_string is defined outside the code block, the last time is it used in code is inside the code block, so this is not a problem for the compiler.
Two references with different lifetimes
Let’s consider this real-world example involving validating and returning a config field. Let’s imagine you’re working in a system that:
- Accepts a configuration object (long-lived)
- Accepts a temporary validation message (short-lived)
- Logs the message
- Returns a reference to the
config.title
field
struct Config {
title: String,
max_connections: usize,
}
fn get_config_title<'a, 'b>(config: &'a Config, validation_note: &'b str) -> &'a str {
println!("Validation note: {}", validation_note);
&config.title
}
fn main() {
let config = Config {
title: "Main Database".to_string(),
max_connections: 100,
};
let title_ref;
{
let note = String::from("Config passed basic validation");
title_ref = get_config_title(&config, ¬e);
}
println!("Config title: {}", title_ref);
}
// Output:
// Validation note: Config passed basic validation
// Config title: Main Database
This code involves a struct with two fields. Our function takes a reference to an instance of the struct, and a string slice for a validation message. The function prints the validation message and returns the title field from the struct instance.
In main() we create an instance of the Config struct, and then in a code block we create the validation message and call our function. Notice that our function signature involves two lifetime annotations 'a
and 'b
:
fn get_config_title<'a, 'b>(config: &'a Config, validation_note: &'b str) -> &'a str
We use lifetime 'a
for the first parameter reference and for the return reference and we use lifetime 'b
for the validation message. This code compiles because the return reference has the same lifetime as the Config instance. If you examine the main() function you will see the Config instance is defined outside the code block but the validation message is defined inside the code block.
We refer to the Config instance reference being “long lived” and the validation message reference being “short lived” because of their lifetimes, how long they live.
Example where this type of code fails
Replace the previous code with the following. This code will not compile.
struct Config {
title: String,
max_connections: usize,
}
fn return_note<'a, 'b>(config: &'a Config, validation_note: &'b str) -> &'b str {
println!("Using config title: {}", config.title);
validation_note
}
fn main() {
let config = Config {
title: "Main Database".to_string(),
max_connections: 100,
};
let note_ref;
{
let note = String::from("This will be returned");
note_ref = return_note(&config, ¬e); // ❌ borrowing from short-lived note
}
println!("Returned note: {}", note_ref); // ⚠️ ERROR: `note` was dropped
}

This code fails because now the function returns the short lived reference of the note but the println!() using the return reference is outside the code block, after the note value has been dropped.
Once again there are at least two possible fixes, move the declaration of the note variable out of the code block, or instead move the println!() into the code block.
Now that we’ve seen how lifetime annotations work in standalone functions, let’s see how they apply to struct methods and associated functions — where lifetimes often appear alongside self
.”
Lifetimes in Structs
In Rust, structs often store references instead of owning their own data. The compiler needs to ensure that those references remain valid for as long as the struct itself exists. Lifetime annotations describe the relationship between the struct’s lifetime and the lifetimes of the references it holds.
Without explicit lifetime annotations, Rust cannot safely compile a struct definition that includes references. In this section, we’ll explore when and why lifetime annotations are needed in structs, and how to work with them across struct definitions and method implementations.
If you need a refresher on Rust structs, this ByteMagma post might help:
Structs in Rust: Modeling Real-World Data
Structs That Hold References
Rust requires lifetime annotations when you define a struct that holds references as fields. These annotations describe how long the data referenced by each field must remain valid — and ensure that the struct doesn’t outlive its borrowed data. Let’s look at how to declare lifetimes on structs and what they mean in practice.
#[derive(Debug)]
struct Person {
name: &str,
age: u8,
}
fn main() {
let frank = Person {
name: "Frank",
age: 34,
};
println!("User name: {:?}", frank.name);
println!("User age: {:?}", frank.age);
}

The error message tells us a lifetime specifier was expected on the struct field that is a reference. It also suggests a fix to the issue. Let’s follow this suggestion and modify our struct definition:
struct Person<'a> {
name: &'a str,
age: u8,
}
Our lifetime annotation for this struct is similar to that for functions. We specify a lifetime after the struct name with <'a>
and we use that lifetime 'a
on the struct field that is a reference.
Now our code compiles fine with this output:
User name: "Frank"
User age: 34
Structs with Nested References
Let’s expand our example to have an Employee struct that has an id field and a person field, both are references:
#[derive(Debug)]
struct Person<'a> {
name: &'a str,
age: u8,
}
struct Employee {
id: &str,
person: &Person,
}
fn main() {
let frank = Person {
name: "Frank",
age: 34,
};
let emp1 = Employee {
id: "001",
person: &frank,
};
println!("Employee id: {:?}", emp1.id);
println!("Employee name: {:?}", emp1.person.name);
println!("Employee age: {:?}", emp1.person.age);
}

This code produces three errors, one about the Employee id reference and two errors about the Employee person reference. The error also provides suggestions for all three. Let’s implement those suggestions. Our code now compiles.
struct Employee<'a> {
id: &'a str,
person: &'a Person<'a>,
}
// Output:
// Employee id: "001"
// Employee name: "Frank"
// Employee age: 34
Although this allows our code to compile, we have a subtle problem here. We specify a lifetime in the Employee struct <'a>
and we use that lifetime specifier on the id field and on the person field.
There are two things to note here:
- The
'a
lifetime for the Employee struct definition is not the same as the'a
lifetime for the Person struct definition. We just happened to use the same label'a
. - But because we do this:
person: &'a Person<'a>
we’re passing down the Employee'a
lifetime into the Person struct, so the'a
lifetime in Person is tightly coupled to the'a
lifetime in the Employee struct.
The following is a better solution:
struct Employee<'a, 'b> {
id: &'a str,
person: &'b Person<'b>,
}
Now we specify two lifetimes for Employee <'a, 'b>
, and Person get’s it’s own lifetime 'b
, distinct and decoupled from lifetime 'a
. We could go even further and use more expressive names for these lifetimes:
struct Person<'pers> {
name: &'pers str,
age: u8,
}
struct Employee<'id, 'p> {
id: &'id str,
person: &'p Person<'p>,
}
Now our code is more expressive and our lifetimes are decoupled. Why does decoupling matter?
1. Overly Restrictive APIs
If your Employee
struct forces the id
and the Person
and the Person
‘s name
to all have the same lifetime 'a
, then you can’t mix data with different lifetimes, even when it’s safe.
You’re reading an id
from a long-lived config, but the Person
data is deserialized from a short-lived HTTP request.
id: &'a str
lives for the entire program ('static
)person: &'a Person<'a>
is temporary, lives just for this request
But Rust forces 'a
to be the same for both. Now you can’t even build the struct, even though your use case is perfectly safe.
2. Harder Borrow Checker Conflicts
By tying all the lifetimes together, the borrow checker is more likely to throw errors when you try to use different parts of your data in different scopes.
If Employee<'a>
keeps references to both id
and Person<'a>
, and you later want to use id
beyond the lifetime of person
, you can’t — even if the id
reference would still be valid.
3. No Room for Partial Struct Initialization or Replacement
With tight coupling, replacing a single field (like person
) with a shorter-lived value could invalidate the whole struct.
Say you’re updating only person
for a short-lived operation, like a temp login. You now can’t reuse the existing Employee
unless all fields fit the same lifetime.
4. Testing and Mocking Become More Painful
In tests, you might want to mix and match:
- Long-lived test fixtures
- Short-lived test data (e.g., inline
&str
literals)
If lifetimes are tightly bound, you can’t easily substitute test inputs with varying lifetimes, making mocks harder to write.
Bottom line – try to avoid passing down lifetimes to nested references, unless you have a very good reason to do so.
Now let’s look at using lifetimes in methods a struct implements.
Implementing Methods for Structs with Lifetimes
Once a struct holds references, any methods that use those fields must also respect the same lifetimes. This includes methods defined in impl
blocks, especially those that take &self
or &mut self
. We’ll see how lifetime annotations carry over into method signatures — and how Rust’s lifetime elision rules can sometimes simplify the syntax for you.
Basic Struct with Reference + Method Returning a Field (Explicit Lifetime)
struct Message<'a> {
content: &'a str,
}
impl<'a> Message<'a> {
fn get_content(&self) -> &'a str {
self.content
}
}
fn main() {
let msg = String::from("Welcome back!");
let message = Message { content: &msg };
println!("Message content: {}", message.get_content());
}
// Output:
// Message content: Welcome back!
Note that we use the same 'a
for the lifetime in the struct definition and the struct impl. These refer to the same lifetime, and this is necessary. The methods implemented on the struct must refer to the same data as the struct.
If you did this:
struct Message<'a> {
content: &'a str,
}
impl<'b> Message<'b> {
fn get_content(&self) -> &'b str {
self.content
}
}
This is technically allowed, but confusing because this compiles only because 'b
= 'a
in context — it’s not a new or different lifetime, it’s just a renamed reuse of the same lifetime from the struct.
'b
is just an alias for 'a
— but it’s confusing, and it makes your code look like it’s using a new lifetime, when it’s really just using the one from the struct. This is valid Rust, but not idiomatic or recommended.
Same Method with Elided Lifetime (elision rule #3, &self
)
struct Message<'a> {
content: &'a str,
}
impl<'a> Message<'a> {
fn get_content(&self) -> &str {
self.content
}
}
fn main() {
let msg = String::from("System rebooting...");
let message = Message { content: &msg };
println!("Content: {}", message.get_content());
}
The only thing we’ve changed here is we have removed the explicit lifetime specifier from the method return type:
fn get_content(&self) -> &str {
This compiles because Rust applies elision rule #3:
“If a method has a parameter &self
or &mut self
, the output gets the same lifetime as self
.“
so when a method takes &self
, the return value’s lifetime is inferred to match. This is another example of the Rust elision rules making our job easier, with less to think about.
Method accepting another input reference, no return value
In the previous example, our struct impl method returned a reference to one of the struct’s fields, and Rust’s elision rules allowed us to omit lifetime annotations.
In this next example, we define a method that takes another reference as a parameter, but doesn’t return a reference. Because we’re not returning any borrowed data, Rust doesn’t require any lifetime annotations.
struct Message<'a> {
content: &'a str,
}
impl<'a> Message<'a> {
fn is_prefix(&self, prefix: &str) -> bool {
self.content.starts_with(prefix)
}
}
fn main() {
let msg = String::from("Processing payment...");
let message = Message { content: &msg };
if message.is_prefix("Processing") {
println!("Message is processing something.");
} else {
println!("Different message.");
}
}
// Output:
// Message is processing something.
This Example Demonstrates:
- A method with
&self
(borrowing the struct) - An additional input reference (
prefix: &str
) - No return of any reference — just a boolean
- No lifetime annotations needed, even though multiple references are involved
As long as you’re not returning a reference, Rust doesn’t care how long the input references live relative to each other — it only checks that they’re valid during the function call. That’s why no lifetime annotations are needed here.
Returning a Field from Struct with Multiple References
In this example, we move from a struct with a single reference field to a struct that holds multiple references, each with the same lifetime (first and second).
The longer()
method returns one of the two references, depending on their length. Even though the method is returning a reference, we don’t need any explicit lifetime annotations in the method signature — Rust can infer them using elision rule #3, because the method takes &self
.
struct Pair<'a> {
first: &'a str,
second: &'a str,
}
impl<'a> Pair<'a> {
fn longer(&self) -> &str {
if self.first.len() > self.second.len() {
self.first
} else {
self.second
}
}
}
fn main() {
let one = String::from("Short");
let two = String::from("Much longer string");
let pair = Pair {
first: &one,
second: &two,
};
println!("Longer string: {}", pair.longer());
}
// Output:
// Longer string: Much longer string
This example demonstrates:
- How to handle multiple borrowed fields in a struct
- That elision still works even when the method has to choose between multiple references
- The return value’s lifetime is tied to
self
, which means it’s guaranteed to be safe as long as thePair
instance is alive
Rust’s lifetime elision rules are powerful enough to handle common patterns like this. Even though we’re returning one of two borrowed fields, the compiler knows that both are valid for the lifetime of the struct — so no explicit lifetimes are required in the method signature. No lifetime annotations needed in the method because &self
elision still applies.
Returning a Reference with Explicit Lifetime Relationships
In this final example, we go beyond the common elision cases. Here, the method receives two references with potentially different lifetimes: one from self
(the struct’s internal reference) and one from an external input (other
).
Because we’re returning a reference to self.content
, we need to explicitly tell the compiler that the returned value will have the same lifetime as self
, not the shorter-lived input. Elision rules can’t handle this case — so we must annotate lifetimes manually.
struct Message<'a> {
content: &'a str,
}
impl<'a> Message<'a> {
fn longest<'b>(&'a self, other: &'b str) -> &'a str {
if self.content.len() >= other.len() {
self.content
} else {
// In a real-world case, you'd return `other` only if it lived as long as `'a`
// Here we just return `self.content` for illustration
self.content
}
}
}
fn main() {
let saved = String::from("Hello, user.");
let message = Message { content: &saved };
let temp = String::from("Hi");
let result = message.longest(&temp);
println!("Longest: {}", result);
}
// Output:
// Longest: Hello, user.
Notice that the struct and impl block have lifetime specifier 'a
and the method longest() has lifetime specifier 'b
. But the two method parameters use different lifetime specifiers. One uses the 'a
specifier from the struct/impl block, and the other uses the method 'b
specifier.
This example demonstrates:
- How to write a method that takes multiple references with different lifetimes
- How to explicitly tie the return value’s lifetime to
self
, and not to other parameters - Why lifetime elision doesn’t apply when multiple lifetimes are involved and the return lifetime isn’t obvious
This example shows the moment when lifetime annotations become truly necessary. When inference can’t help — especially in functions or methods that take multiple input references — it’s up to us to tell the compiler how those lifetimes relate. That’s where explicit lifetime annotations shine.
Structs with Multiple Lifetimes
Sometimes a struct might hold references to different sources of data, each with its own lifetime. In these cases, a single lifetime annotation isn’t enough — we need to introduce multiple lifetimes and explain how they relate to the struct’s fields.
Imagine you’re working with data from two different sources:
- A title from a long-lived config file
- A body from a user request that lives shorter
Here’s how to design a struct to handle this. Each field has its own lifetime. The compiler ensures both references are valid for as long as the Record
exists — but they don’t need to share the same lifetime.
struct Record<'title, 'body> {
title: &'title str,
body: &'body str,
}
impl<'title, 'body> Record<'title, 'body> {
fn summary(&self) -> &str {
if self.title.len() > self.body.len() {
self.title
} else {
self.body
}
}
}
fn main() {
let config_title = String::from("System Alert");
let user_input = String::from("Low disk space on volume /dev/sda1.");
let record = Record {
title: &config_title,
body: &user_input,
};
let summary_result = record.summary(); // safe: both live long enough
println!("Summary: {}", summary_result);
}
// Output:
// Summary: Low disk space on volume /dev/sda1.
This example demonstrates:
- You can create a struct that mixes data from sources with different lifetimes
- The compiler enforces that all borrowed data lives long enough
- You must annotate the struct with one lifetime per field that holds a reference
Constructor Function with Explicit Lifetimes
Here is an example of an associated function, a constructor for the Record struct.
impl<'title, 'body> Record<'title, 'body> {
fn new(title: &'title str, body: &'body str) -> Self {
Record { title, body }
}
}
You can call it like this:
let record = Record::new(&config_title, &user_input);
Note that associated functions — that is, functions in an impl
block that don’t take self
— follow the same lifetime rules as regular functions. If they take or return references, you’ll often need to annotate lifetimes explicitly. Since they don’t benefit from &self
elision rules, the syntax might feel a bit more verbose — but the rules you’ve already learned apply exactly the same.
When to Avoid References in Structs
There are situations where using references in structs makes sense — and others where it’s better to avoid them. Here we offer guidance on when to use owned data instead of references, and how techniques like String
over &str
can simplify your lifetime management and reduce annotation overhead.
With references (requires lifetime annotations):
struct UserView<'a> {
username: &'a str,
email: &'a str,
}
- Requires lifetime parameters on the struct
- Restricts how long you can use the struct (tied to the borrowed data)
With owned data (simpler, more flexible):
struct UserOwned {
username: String,
email: String,
}
- No lifetime annotations
- Easier to store, clone, move between threads, etc.
If your struct owns its data (e.g., using String
instead of &str
), you eliminate the need for lifetime annotations entirely. This often makes your code more flexible and easier to use — especially if you’re storing the struct, sending it between threads, or returning it from functions.
As a rule of thumb: use owned types (String
, Vec<T>
, etc.) unless you have a compelling reason to borrow. Lifetimes add power, but they also add complexity — and in many real-world scenarios, owned data makes development faster, safer, and more ergonomic.
Note on Enums
Enums that hold references work just like structs — if any variant contains a reference, the enum needs a lifetime annotation. Matching on such enums or returning references from them follows the same rules as working with structs with lifetimes.
Lifetimes and Generics
Lifetimes and generics often go hand-in-hand in Rust. When you’re working with generic functions, structs, or enums that involve references, you’ll often need to annotate both type parameters and lifetimes. In this section, we’ll explore how to combine lifetimes with generics, how to write generic code that involves references, and how to apply lifetime bounds correctly to ensure your code is both safe and flexible.
If you need a refresher on Rust generics, this ByteMagma post might help:
Generics in Rust: Writing Flexible and Reusable Code
Generic Functions with Lifetimes
When writing generic functions that work with references, lifetimes help the compiler ensure that the data you’re borrowing remains valid. In simple cases, Rust can infer lifetimes using its elision rules — but as soon as multiple lifetimes or returned references are involved, you’ll need to specify lifetime relationships explicitly.
Let’s look at common patterns involving generic functions and lifetimes, including how to annotate function parameters, how to safely return references, and when you can rely on elision to keep your code clean.
Function Parameters with Generic Types and Lifetimes
Generic functions often take references to generic types — for example, &T
— and when those references must live for a particular lifetime, you need to annotate accordingly.
fn print_label<'a, T: std::fmt::Display>(label: &'a T) {
println!("Label: {}", label);
}
fn main() {
let name = String::from("Processor Unit");
print_label(&name);
}
// Output:
// Label: Processor Unit
This function takes a reference to a generic T
that implements Display
, and it’s valid for lifetime 'a
. We’re not returning anything, so the lifetime is used only to ensure the reference is valid for the duration of the call.
Note that this function does not need lifetime annotations because there is only one input reference and the function does not return a reference.
In simple generic functions that only accept references and don’t return any, lifetime annotations are usually unnecessary thanks to Rust’s elision rules. The compiler knows the reference only needs to be valid for the duration of the call.
Returning References from Generic Functions
When a function both takes references and returns one, Rust needs to know the relationship between input and output lifetimes. Here’s a real-world example that returns the longer of two string-like inputs.
fn longer_string<'a, T: AsRef<str>>(str_one: &'a T, str_two: &'a T) -> &'a str {
let one = str_one.as_ref();
let two = str_two.as_ref();
if one.len() > two.len() {
one
} else {
two
}
}
fn main() {
let s1 = String::from("Database");
let s2 = String::from("Cache");
let result = longer_string(&s1, &s2);
println!("Longer label: {}", result);
}
// Output:
// Longer label: Database
This generic function works with any type that can be viewed as a string (AsRef<str>
) and returns a reference tied to the input lifetime 'a
. You’re returning a &str
, which is derived from either str_one or str_two
— both of which are references. So Rust needs to know how long the returned reference is valid for.
By writing -> &'a str
, you’re saying:
“The returned string slice is guaranteed to be valid as long as 'a
— the same lifetime as the input references str_one and str_two.”
Note that AsRef<str>
is a standard library trait that means:
“I don’t care what type T
is, as long as it can be converted into a &str
.”
This allows the function to work with String
, &str
, and any custom type that implements AsRef<str>
.
You’re calling .as_ref()
because T
is a generic type, and you’ve constrained it with:
T: AsRef<str>
So even though str_one
and str_two
are references (i.e., &T
), Rust doesn’t know that T
is already a &str
. It only knows that T
can produce a &str
— via as_ref()
.
Lifetime Elision vs. Explicit Annotations in Generic Contexts
As we’ve seen, Rust’s lifetime elision rules often make explicit annotations unnecessary — especially when functions only take a single reference input or don’t return references.
Here’s an example where we do return a reference, and Rust can still elide the lifetime:
fn echo<T>(input: &T) -> &T {
input
}
fn main() {
let warning = "Overheating!";
let repeated = echo(&warning);
println!("Echo: {}", repeated);
}
// Output:
// Echo: Overheating!
Rust applies elision rule #2 here: if a function has exactly one input reference, the return reference gets that same lifetime. Since there’s no ambiguity, no annotations are needed.
If you added a second reference, though, Rust would require you to annotate explicitly — because it could no longer infer which reference the return value was tied to.
- With one input reference, Rust can infer the output lifetime — but with multiple inputs or more complex constraints, you’ll need to specify lifetimes explicitly.
- Combining generics and lifetimes is about precision: you’re telling Rust exactly how the lifetimes of references relate to each other across types.
Generic Structs with Lifetimes
When designing generic structs in Rust, you often want to combine type parameters with references. This adds a new layer of complexity — you not only have to track how generic types are used, but also how long the references inside your struct live. That’s where lifetime parameters come in.
Let’s look at how to define structs that are both generic and lifetime-aware, how to mix owned and borrowed fields effectively, and how lifetime requirements can influence how generics are used and constrained.
Structs with Both Type Parameters and Lifetime Parameters
When a struct holds a reference to a generic type, Rust needs to know how long that reference is valid. That means adding both a type parameter (T
) and a lifetime parameter ('a
).
struct Wrapper<'a, T> {
value: &'a T,
}
fn main() {
let data = String::from("Cached response");
let wrapper = Wrapper { value: &data };
println!("Wrapped value: {}", wrapper.value);
}
// Output:
// Wrapped value: Cached response
This struct can hold a reference to any T
for the value field, as long as that value field reference lives at least as long as the lifetime of the struct 'a
.
Field Combinations: Owned Types, Borrowed Types, Mixed Types
Sometimes you want your struct to own some data and borrow other parts — for flexibility, performance, or design reasons. Here’s an example of a struct with both owned and borrowed fields:
struct Document<'a> {
id: u32,
title: String,
body: &'a str,
}
fn main() {
let body_text = String::from("Rust lifetimes are powerful and safe.");
let doc = Document {
id: 42,
title: "Learning Lifetimes".to_string(),
body: &body_text,
};
println!("Doc #{}: {}", doc.id, doc.body);
}
// Output:
// Doc #42: Rust lifetimes are powerful and safe.
Our Document struct has two fields that are owned data and one field body that data borrowed with a reference. This is a common real-world pattern — owning things like IDs or titles (which are cheap or come from other systems), while borrowing large text blobs to avoid unnecessary cloning.
Lifetime-Driven Constraints on Generic Fields
Sometimes the generic type itself must contain references, and you need to ensure those references are valid for a particular lifetime. That’s when you add lifetime bounds on the type parameter, like T: 'a
.
struct CacheEntry<'a, T: 'a> {
key: &'a str,
value: T,
}
fn main() {
let key = "session_token";
let value = String::from("abc123");
let entry = CacheEntry {
key,
value,
};
println!("Cache: {} => {}", entry.key, entry.value);
}
// Output:
// Cache: session_token => abc123
Here, T: 'a
means that the type T
must not contain references that outlive 'a
. If T
does contain references, the compiler will verify that they also live at least as long as 'a
.
This struct has:
- A lifetime parameter:
'a
- A type parameter:
T
- A field
key
that is a&'a str
— a reference that must live at least as long as'a
- A field
value
of typeT
- A lifetime bound on the type:
T: 'a
What does T: 'a
mean?
This is a lifetime bound
on the generic type T
.
It tells the compiler:
“If T
contains any references internally, those references must live at least as long as 'a
.”
In other words, T
may contain references — and if it does, those references cannot outlive 'a
.
Why is this necessary?
Because CacheEntry<'a, T>
is storing:
- A reference
key: &'a str
- A value of type
T
If T
also contains any internal references, and you don’t specify T: 'a
, the compiler has no way of verifying that those references won’t be dropped too soon. You’re asking Rust to ensure the entire struct is valid for at least 'a
, so everything inside must uphold that guarantee — including the internals of T
.
This pattern is common when building caches, wrappers, or adapters that store generic data but need to reference keys or metadata with a defined lifetime.
- Generic structs that hold references need lifetime annotations to tell Rust how long the borrowed data must live.
- You can mix owned and borrowed fields freely — as long as you annotate lifetimes correctly.
- Lifetime bounds like
T: 'a
are necessary when the generic type may itself contain references tied to your struct’s lifetime.
Lifetime Bounds in Generic Implementations
Let’s look a bit more closely at lifetime bounds with generics.
When implementing traits or methods for generic types, sometimes the generic type itself may contain references. In those cases, Rust needs to know how long those references live. That’s where lifetime bounds come into play.
A lifetime bound like T: 'a
means that any references inside the type T
must live at least as long as 'a
. Without this constraint, the compiler can’t guarantee safety — especially when the generic value is stored alongside other references or returned from a method.
Let’s see how to write implementations that include lifetime bounds, and when to use them directly in the impl
header or with a where
clause for more clarity and flexibility.
impl<'a, T> Struct<T>
vs.
impl<T: 'a> Struct<T>
These two forms look similar but behave differently:
impl<'a, T>
simply introduces a lifetime'a
and a typeT
— but says nothing about howT
and'a
relateimpl<T: 'a>
adds a constraint: it says that ifT
contains references, they must live at least as long as'a
Implementing a method for types with references
struct LogEntry<'a, T: 'a> {
label: &'a str,
data: T,
}
impl<'a, T: 'a> LogEntry<'a, T> {
fn describe(&self) {
println!("{} => entry recorded", self.label);
}
}
fn main() {
let label = "event";
let description = String::from("System rebooted");
let entry = LogEntry {
label,
data: description,
};
entry.describe();
}
// Output:
// event => entry recorded
We use T: 'a
in the impl
to guarantee that if T
contains references, they won’t outlive 'a
, which is how long the struct promises to be valid.
Applying Lifetime Bounds to Type Parameters
You may encounter this when writing methods that accept or return references to the generic parameter T
.
A wrapper that returns a reference to its data
struct Holder<'a, T: 'a> {
data: &'a T,
}
impl<'a, T: 'a> Holder<'a, T> {
fn get(&self) -> &'a T {
self.data
}
}
fn main() {
let value = 42;
let holder = Holder { data: &value };
let extracted = holder.get();
println!("Held value: {}", extracted);
}
// Output:
// Held value: 42
The lifetime bound T: 'a
ensures that the reference inside data
is valid for 'a
, and that we can safely return a reference to it from the method.
Where Clauses with Lifetimes and Generics
When lifetime bounds get long or complicated, you can move them to a where
clause for better readability and clarity.
struct ConfigEntry<'a, T> {
key: &'a str,
value: T,
}
impl<'a, T> ConfigEntry<'a, T>
where
T: std::fmt::Display + 'a,
{
fn show(&self) {
println!("{} => {}", self.key, self.value);
}
}
fn main() {
let key = "timeout";
let value = 30;
let entry = ConfigEntry {
key,
value,
};
entry.show();
}
// Output:
// timeout => 30
Here, the where
clause keeps the impl
clean and tells the compiler: “The type T
must be printable, and any references inside it must live at least as long as 'a
.”
- Use
T: 'a
when your generic type might contain references that must be valid for'a
- Use
impl<'a, T: 'a>
when implementing for structs that combine both lifetimes and generics - Use
where
clauses when your lifetime bounds grow complex or when you want to separate constraints from the type declaration
Avoiding Common Pitfalls with Lifetimes and Generics
- Overusing
'static
when a shorter lifetime is sufficient (we’ll cover the'static
lifetime soon) - Misplacing lifetime parameters in generic signatures
- Troubleshooting compiler errors in generic lifetime contexts
Lifetimes and Traits
When working with traits in Rust, lifetimes can surface in both expected and subtle ways. Traits that work with references often require lifetime annotations, and things become more complex when traits are used as bounds, implemented for references, or combined with generics and trait objects. Let’s explores how lifetimes interact with traits across definitions, implementations, and usage — and where explicit annotations are necessary to ensure safety and clarity.
Sometimes a trait needs to work with references — whether that’s in method parameters, return values, or associated data. In those cases, you must add lifetime parameters to the trait definition itself.
This lets the compiler enforce how long the trait’s references must live — and it also enables you to implement the trait for types that carry lifetimes, like references or lifetime-annotated structs.
Let’s explore how to define lifetime-aware traits, use them in method signatures, and understand how those lifetimes affect trait implementations.
Trait with Reference Arguments
Traits often define methods that take references as arguments. If the reference needs to live beyond the function scope (e.g., it’s stored or reused), the trait must carry a lifetime.
trait Greeter<'a> {
fn greet(&self, name: &'a str);
}
struct Console;
impl<'a> Greeter<'a> for Console {
fn greet(&self, name: &'a str) {
println!("Hello, {}!", name);
}
}
fn main() {
let user = String::from("Greg");
let console = Console;
console.greet(&user);
}
// Output:
// Hello, Greg!
The trait Greeter<'a>
means that any type implementing the trait must define a greet
method that accepts a reference which is valid for the lifetime 'a
.
Trait with Reference Return Types
If a trait method returns a reference, you need to declare the lifetime of that return value in the trait signature.
trait Finder<'a> {
fn find(&self) -> &'a str;
}
struct ErrorSource<'a> {
message: &'a str,
}
impl<'a> Finder<'a> for ErrorSource<'a> {
fn find(&self) -> &'a str {
self.message
}
}
fn main() {
let msg = String::from("Disk not found");
let source = ErrorSource { message: &msg };
println!("Error: {}", source.find());
}
// Output:
// Error: Disk not found
The 'a
lifetime on the trait tells the compiler the reference returned from trait method find() must remain valid as long as the struct’s borrowed data does, in this example the message field of ErrorSource.
Trait with Lifetime Parameters on the Trait Itself
Sometimes, the trait doesn’t just accept references — it models behavior over the course of a lifetime. In that case, the trait itself carries a lifetime parameter, and it’s applied across all methods.
trait Loggable<'a> {
fn category(&self) -> &'a str;
fn message(&self) -> &'a str;
}
struct Event<'a> {
category: &'a str,
message: &'a str,
}
impl<'a> Loggable<'a> for Event<'a> {
fn category(&self) -> &'a str {
self.category
}
fn message(&self) -> &'a str {
self.message
}
}
fn main() {
let cat = String::from("system");
let msg = String::from("Startup complete");
let event = Event {
category: &cat,
message: &msg,
};
println!("[{}] {}", event.category(), event.message());
}
// Output:
// [system] Startup complete
In this example, the trait Loggable<'a>
defines a contract for types that return references to data that must live at least as long as 'a
. The lifetime 'a
is applied to the return types of all trait methods, meaning any implementor must guarantee that the returned references remain valid for the lifetime 'a
.
This is useful when you want to express that the data being exposed by the trait — such as log message content — is borrowed rather than owned, and you need that borrowed data to remain valid for some externally defined lifetime.
In our case, Event<'a>
stores two string slices that must live at least as long as 'a
, and its Loggable<'a>
implementation simply returns those slices. By tying the lifetime to the trait, we ensure that all consumers of the trait can rely on a consistent lifetime contract across methods.
- It’s especially important when returning references or storing them inside types that implement the trait.
- If your trait works with references — either as inputs or outputs — you’ll usually need to add a lifetime parameter to the trait itself.
- This lifetime gets propagated through the trait’s method signatures and ensures all implementors respect those reference lifetimes.
Implementing Traits for Types with Lifetimes
When a type contains references, any traits implemented for that type must also respect those lifetimes. This means that both the trait definition and the impl
block may need lifetime annotations to ensure references remain valid and safe to use.
Let’s look at how to implement traits for:
- References themselves (e.g.,
&str
,&T
) - Structs that contain references
- Generic implementations that include lifetime bounds
Each example will highlight how lifetime annotations flow through trait implementations and how they influence method signatures.
Implementing Traits for References
Traits can be implemented for reference types directly. This is useful when you want a trait to apply to borrowed data — like &str
or &T
— instead of requiring ownership.
trait TrimAndDisplay {
fn trimmed(&self) -> &str;
}
impl TrimAndDisplay for &str {
fn trimmed(&self) -> &str {
self.trim()
}
}
fn main() {
let raw = " Rustacean ";
let trimmed = raw.trimmed();
println!("Trimmed: '{}'", trimmed);
}
// Output:
// Trimmed: 'Rustacean'
This implementation works on any &str
without requiring ownership, and no explicit lifetime annotations are needed because Rust’s lifetime elision rules apply: the reference in self
gets an implicit lifetime, and the returned reference is inferred to have the same lifetime as self
.
Implementing Traits for Structs with Lifetimes
When implementing a trait for a struct that holds references, both the impl
and the method signatures must reflect the lifetime of the struct.
trait Reporter {
fn report(&self) -> &str;
}
struct Alert<'a> {
message: &'a str,
}
impl<'a> Reporter for Alert<'a> {
fn report(&self) -> &str {
self.message
}
}
fn main() {
let msg = String::from("Battery low");
let alert = Alert { message: &msg };
println!("Report: {}", alert.report());
}
// Output:
// Report: Battery low
Because Alert
holds a reference with lifetime 'a
, the impl
block must reflect that, and the trait method must return a reference tied to that lifetime.
Implementing Traits with Lifetime Bounds on Methods
Sometimes a trait doesn’t require a lifetime on the trait itself, but the implementation for a specific type does — especially when returning references tied to input arguments or struct fields.
trait Describable {
fn describe(&self) -> &str;
}
struct Log<'a> {
content: &'a str,
}
impl<'a> Describable for Log<'a> {
fn describe(&self) -> &str {
self.content
}
}
fn main() {
let text = String::from("System initialized.");
let log = Log { content: &text };
println!("Description: {}", log.describe());
}
// Output:
// Description: System initialized.
Even though Describable
has no lifetime, the impl
and method must still be annotated to ensure that the returned reference is valid for 'a
.
This pattern is common when using third-party traits that can’t be changed to include lifetimes, but you still need to provide safe implementations for types with borrowed data.
- Rust’s lifetime elision rules can reduce verbosity, but when in doubt, be explicit — especially when returning borrowed data.
- When implementing traits for reference types or structs with references, your
impl
blocks and method signatures must reflect those lifetimes. - Even if the trait doesn’t define a lifetime, your implementation must uphold Rust’s safety guarantees by correctly annotating return values and input parameters.
Traits and Lifetime Bounds
When working with traits in Rust, you often need to express not just what a type can do (via trait bounds), but also how long references inside that type must live. This is where lifetime bounds like T: 'a
come into play.
A lifetime bound such as T: 'a
tells the compiler that any references contained inside T
must live at least as long as 'a
. This is critical when combining lifetimes with traits — whether you’re implementing traits, accepting trait-bounded parameters, or building reusable abstractions.
Let’s explore how to express lifetime bounds in trait contexts, how subtyping affects them, and how where
clauses help keep your code readable.
Adding Lifetime Bounds to Generic Type Parameters
When a generic type parameter may contain references, and you want those references to be valid for a specific lifetime, you use a bound like T: 'a
.
fn print_message<'a, T>(msg: &'a T)
where
T: std::fmt::Display + 'a,
{
println!("Message: {}", msg);
}
fn main() {
let content = String::from("Low memory warning");
print_message(&content);
}
// Output:
// Message: Low memory warning
Here, T: 'a
ensures that if T
contains references internally, they live at least as long as 'a
. The compiler enforces this to ensure msg
remains valid during the function call.
This pattern shows up in trait-bound generic functions, especially when data is borrowed across scopes or returned.
Lifetime Bounds in where
Clauses
Lifetime bounds can make type declarations long and hard to read — especially when multiple trait bounds are involved. In these cases, a where
clause improves clarity.
fn save_to_log<'a, T>(entry: &'a T)
where
T: std::fmt::Debug + 'a,
{
println!("Logged entry: {:?}", entry);
}
fn main() {
let record = String::from("2024-04-15: Service started.");
save_to_log(&record);
}
// Output:
// Logged entry: "2024-04-15: Service started."
The where
clause cleanly separates the trait and lifetime bounds, making the signature easier to read and scale — especially when more constraints are added later.
Lifetime Subtyping and Variance in Trait Bounds
Rust allows one lifetime to be a subtype of another — meaning it lives at least as long. This allows more flexible function signatures, especially with generic trait objects and references.
fn longest<'short, 'long: 'short>(a: &'short str, b: &'long str) -> &'short str {
if a.len() > b.len() {
a
} else {
&b[..a.len()] // trim to match 'short lifetime
}
}
fn main() {
let short = String::from("Hi");
let long = String::from("Hello, Greg!");
let result = longest(&short, &long);
println!("Truncated: {}", result);
}
// Output:
// Truncated: He
The bound 'long: 'short
means 'long
must live at least as long as 'short
. This enables the returned reference to be safely tied to the shorter of the two lifetimes, ensuring no invalid data is returned.
This kind of lifetime subtyping comes up frequently when building safe APIs that work with borrowed data of different durations.
- Use
T: 'a
when your type parameter might contain references that need to outlive'a
- Use
where
clauses to express lifetime and trait bounds cleanly - Understand subtyping (
'long: 'short
) to write more flexible and safe APIs that return references
Lifetimes with Traits and Generics
When writing generic code in Rust, you often need to combine trait bounds with lifetime bounds to ensure that borrowed data remains valid and trait constraints are respected. These lifetime + trait combos show up everywhere — from generic functions and structs to Box<dyn Trait + 'a>
and T: Trait + 'static
.
This section focuses on how to build flexible, lifetime-safe abstractions using generic types, trait bounds, and explicit lifetimes together.
Lifetime Bounds on Generic Parameters
You use T: 'a
to express that any references inside T
must live at least as long as 'a
— a constraint that’s essential when borrowing data tied to a lifetime.
fn print_debug<'a, T>(val: &'a T)
where
T: std::fmt::Debug + 'a,
{
println!("Debug: {:?}", val);
}
fn main() {
let data = String::from("loaded config");
print_debug(&data);
}
// Output:
// Debug: "loaded config"
This bound ensures that T
doesn’t contain any references that might expire before 'a
, keeping val
valid throughout the call.
Combining Trait Bounds and Lifetime Bounds
It’s common to require both a trait and a lifetime on a generic parameter — for example, when formatting borrowed data.
fn display_label<'a, T>(label: &'a T)
where
T: std::fmt::Display + 'a,
{
println!("Label: {}", label);
}
fn main() {
let title = String::from("Service Status");
display_label(&title);
}
// Output:
// Label: Service Status
T: Display + 'a
means we can both format the type and guarantee that any references inside it live at least as long as 'a
.
You’ll see this often in generic wrappers, loggers, formatters, and error reporting utilities.
Lifetimes in Generic Trait Implementations
When implementing traits for generic types, lifetimes often need to be included — especially if the implementation relies on borrowed data.
trait Announce {
fn headline(&self) -> &str;
}
struct Notice<'a, T> {
source: &'a str,
message: T,
}
impl<'a, T> Announce for Notice<'a, T>
where
T: std::fmt::Display,
{
fn headline(&self) -> &str {
self.source
}
}
fn main() {
let label = "⚠️ Alert";
let msg = String::from("High memory usage detected.");
let notice = Notice {
source: label,
message: msg,
};
println!("From {}: {}", notice.headline(), notice.message);
}
// Output:
// From ⚠️ Alert: High memory usage detected.
The struct uses a generic type T
and a reference with lifetime 'a
, and the trait implementation leverages both.
This is a practical example of writing reusable trait impls that still respect Rust’s strict borrowing rules.
Lifetime Bounds in Trait Objects
When you create trait objects, you may need to specify a lifetime to ensure the reference to the trait object remains valid. For example, &'a dyn Trait
or Box<dyn Trait + 'a>
.
trait Command {
fn execute(&self);
}
struct Shutdown;
impl Command for Shutdown {
fn execute(&self) {
println!("System shutting down...");
}
}
fn run_command<'a>(cmd: &'a dyn Command) {
cmd.execute();
}
fn main() {
let shutdown = Shutdown;
run_command(&shutdown);
}
// Output
// System shutting down...
Here, &'a dyn Command
means the trait object must live at least as long as 'a
. This avoids dangling trait object references — especially when trait objects are stored in containers or passed between threads.
Avoiding Over-Constraining with 'static
A common mistake is using T: 'static
by default, which requires that T
(and any references inside it) live for the entire program — often stricter than necessary.
fn send_event<'a, T>(event: &'a T)
where
T: std::fmt::Debug + 'a,
{
println!("Sending event: {:?}", event);
}
fn main() {
let msg = String::from("Session expired.");
send_event(&msg);
}
// Output:
// Sending event: "Session expired."
This avoids forcing 'static
, and instead uses a more precise lifetime 'a
that matches the actual borrowed data’s lifetime.
Only use 'static
when your data really does live forever — like global constants or long-lived background threads.
- Trait objects like
&'a dyn Trait
orBox<dyn Trait + 'a>
require lifetime annotations to ensure safe use across scopes or threads - Use
T: Trait + 'a
to combine trait capabilities and lifetime constraints - When returning or storing references, be precise with your lifetime bounds — don’t default to
'static
Lifetimes in Trait Objects
Trait objects (dyn Trait
) let you work with types through dynamic dispatch, enabling polymorphism without generics. But when using references to trait objects, you must also think about how long those references remain valid — and that’s where lifetimes in trait objects come in.
Rust requires you to explicitly annotate the lifetime of a trait object reference when the compiler cannot infer it safely. Whether you’re using &'a dyn Trait
or Box<dyn Trait + 'a>
, these annotations ensure the borrowed trait object doesn’t outlive the data it may refer to — and help you avoid unsound behavior in cases involving borrowing, async, or interior mutability.
Let’s see how trait object lifetimes work, when to annotate them, and how 'static
fits into the picture.
Trait Objects with References
When a trait method returns a reference, or the trait object is borrowed, you need to annotate its lifetime explicitly.
trait Description {
fn describe(&self) -> &str;
}
struct Alert {
message: String,
}
impl Description for Alert {
fn describe(&self) -> &str {
&self.message
}
}
fn print_description(desc: &dyn Description) {
println!("-> {}", desc.describe());
}
fn main() {
let alert = Alert {
message: "CPU temperature high".to_string(),
};
print_description(&alert);
}
// Output:
// -> CPU temperature high
In this case, the compiler can infer the lifetime of &dyn Description
from usage. But if the trait object escapes a local scope or gets stored, you’ll need to annotate the lifetime explicitly.
Using dyn Trait + 'a
Syntax
To store or return trait objects safely, you often need to specify how long the trait object reference must live — using dyn Trait + 'a
.
trait Logger {
fn log(&self);
}
struct Console;
impl Logger for Console {
fn log(&self) {
println!("Logged to console");
}
}
fn get_logger<'a>() -> Box<dyn Logger + 'a> {
Box::new(Console)
}
fn main() {
let logger = get_logger();
logger.log();
}
// Output:
// Logged to console
Here, Box<dyn Logger + 'a>
explicitly sets the trait object’s lifetime. The compiler ensures that the Box
and anything inside it are valid for 'a
. This is critical when returning trait objects from functions.
When Explicit Lifetime Bounds Are Required
When storing a trait object inside another struct or passing it between threads or async tasks, Rust often cannot infer the lifetime and forces you to write it explicitly.
trait Processor {
fn process(&self);
}
struct Task<'a> {
job: &'a dyn Processor,
}
struct PrintJob;
impl Processor for PrintJob {
fn process(&self) {
println!("Processing print job...");
}
}
fn main() {
let job = PrintJob;
let task = Task { job: &job };
task.job.process();
}
// Output:
// Processing print job...
Without the
<'a>
onTask
, this would not compile. Rust needs to know how longjob
will live, and'a
ties the lifetime of the trait object to the struct that holds it.
- When using
dyn Trait
, the reference or container holding the trait object must be tied to a lifetime - Use
&'a dyn Trait
orBox<dyn Trait + 'a>
to be explicit when storing or returning trait objects - The compiler often needs help inferring trait object lifetimes, especially when:
- Returning trait objects
- Passing them between threads
- Storing them inside structs or async tasks
Lifetimes in Supertraits and Associated Types
As you move into more advanced trait design in Rust, you’ll encounter supertraits (traits that require other traits) and associated types (types defined inside a trait). Both of these features can involve lifetimes — and when they do, things get a bit more intricate.
You may want to:
- Require that an implementor of your trait also implement another trait that involves lifetimes
- Define associated types that are references or contain references
- Use those associated types in method signatures that must remain lifetime-safe
Let’s look at how to define and implement traits with lifetime-carrying supertraits, and how to declare associated types that borrow data, all while maintaining safety and clarity.
Supertraits with Lifetime Requirements
When a trait requires another trait — a supertrait — and that trait involves a lifetime, you need to propagate the lifetime explicitly.
trait Source<'a> {
fn get(&'a self) -> &'a str;
}
trait Labeled<'a>: Source<'a> {
fn label(&self) -> &'a str;
}
struct Config<'a> {
data: &'a str,
tag: &'a str,
}
impl<'a> Source<'a> for Config<'a> {
fn get(&'a self) -> &'a str {
self.data
}
}
impl<'a> Labeled<'a> for Config<'a> {
fn label(&self) -> &'a str {
self.tag
}
}
fn main() {
let content = String::from("timeout=30");
let tag = String::from("network");
let config = Config {
data: &content,
tag: &tag,
};
println!("Label: {}, Data: {}", config.label(), config.get());
}
// Output:
// Label: network, Data: timeout=30
Labeled<'a>: Source<'a>
means any type that implements Labeled
must also implement Source
, and both share the same lifetime 'a
. This ties all behavior to the same lifetime and avoids mismatched borrowing issues.
Associated Types that Are References
Associated types inside traits can themselves carry lifetimes. You must declare the lifetime on the trait and reference it in the associated type.
trait Storage<'a> {
type Item;
fn fetch(&'a self) -> Self::Item;
}
struct Text<'a> {
line: &'a str,
}
impl<'a> Storage<'a> for Text<'a> {
type Item = &'a str;
fn fetch(&'a self) -> Self::Item {
self.line
}
}
fn main() {
let sentence = String::from("Rust is safe and fast.");
let text = Text { line: &sentence };
println!("Fetched: {}", text.fetch());
}
// Output:
// Fetched: Rust is safe and fast.
Here, the associated type Item
is itself a &'a str
. This allows the trait to expose borrowed values safely and precisely.
This pattern is useful when designing reusable container-like traits that may operate over borrowed values, such as data loaders, caches, or DOM wrappers.
- When using supertraits with lifetimes, all related traits and methods must agree on how long references live
- Associated types can carry lifetimes, and doing so allows traits to return references in a safe and generic way
- Traits that expose borrowed data through methods or associated types require careful lifetime coordination, especially in layered trait designs
Blanket Implementations and Lifetimes
Rust allows you to implement traits for broad sets of types using what’s called a blanket implementation — where you write impl<T> Trait for T
with optional bounds. These impls are incredibly powerful, but when working with lifetimes, they require precision: if the generic type T
contains references, you may need to add lifetime bounds like T: 'a
to ensure safety.
Let’s look at how to safely write blanket impls for types that may contain references, and how to use T: 'a
or trait object bounds to ensure the implementation is valid for all intended lifetimes.
Blanket Impls with Lifetime Bounds
You can constrain a blanket impl so that it only applies to types that are valid for a specific lifetime. This is helpful when your trait methods return references, or your generic type may include borrowed data.
trait Announce {
fn announce(&self) -> &str;
}
impl<'a, T> Announce for &'a T
where
T: std::fmt::Display,
{
fn announce(&self) -> &str {
// Simulated static announcement
"Announcement made."
}
}
fn main() {
let name = String::from("Greg");
let reference = &name;
let msg = reference.announce();
println!("{}", msg);
}
// Output:
// Announcement made.
This impl covers all references to types that implement Display
, and is valid for any lifetime 'a
. It’s simple here, but this pattern is often used to add behavior to all references of a certain kind — useful in traits like Borrow
, ToOwned
, or logging frameworks.
Blanket Impls with T: 'a
Lifetime Constraints
If you’re implementing a trait where the method returns a reference, you’ll need to ensure the returned data is valid. That’s when T: 'a
becomes essential — to guarantee T
’s internals won’t expire too soon.
trait Describable<'a> {
fn describe(&'a self) -> &'a str;
}
impl<'a, T> Describable<'a> for T
where
T: std::fmt::Debug + 'a,
{
fn describe(&'a self) -> &'a str {
// Just a placeholder for blog demo purposes
"Description stub"
}
}
fn main() {
let log = String::from("All systems nominal");
let summary = (&log as &dyn Describable).describe();
println!("Summary: {}", summary);
}
// Output:
// Summary: Description stub
T: 'a
ensures that if T
contains references, they must outlive 'a
. This allows the trait method to safely return a borrowed value (simulated here), tied to the input lifetime.
In more realistic code, you’d return a borrowed field or dynamically built reference from inside T
.
- Blanket impls are powerful, but must be bounded carefully when references are involved
- Use
T: 'a
to ensure that types with internal references live long enough for trait methods to use them - Blanket impls often show up in real-world libraries when extending standard types, references, or smart pointers with new behavior — and lifetime bounds are what make those extensions safe
The static Lifetime
The 'static
lifetime is a special lifetime in Rust that signifies “lives for the entire duration of the program.” While it may sound like something only used in low-level or embedded code, 'static
shows up in many everyday Rust programs — often implicitly. Understanding when 'static
applies, when it’s required, and when it causes confusion is key to writing ergonomic and safe Rust.
Let’s explore how 'static
works, where it appears naturally, and how to use it (or avoid it) effectively.
Using 'static
in Function Signatures
The 'static
lifetime means “this data lives for the entire duration of the program.” While it’s often associated with global constants, you’ll also see 'static
appear in function signatures — particularly in:
- Function parameters, to ensure inputs don’t reference temporary data
- Return values, for long-lived references (e.g. cached strings or global configs)
- Generic trait bounds, where
'static
ensures that types or trait objects don’t include borrowed data
Let’s see how and when to use 'static
in function signatures — and when it might be unnecessary or overly strict.
Explicit 'static
in Input Parameters
You can require that an input reference lives for the entire program — useful when accepting global data or static string slices.
fn announce(msg: &'static str) {
println!("System Message: {}", msg);
}
fn main() {
// string literal has a 'static lifetime
let banner: &'static str = "Launch sequence initiated";
announce(banner);
}
// Output:
// System Message: Launch sequence initiated
In this case, the compiler knows the string literal lives forever, so passing it as &'static str
is safe and natural. This pattern is common in logging systems and low-level frameworks that store or reuse messages indefinitely.
Returning 'static
References
Sometimes, a function may return a reference with a 'static
lifetime — usually when returning a global constant or cached value that never gets deallocated.
fn get_status_label(code: u8) -> &'static str {
match code {
0 => "OK",
1 => "WARN",
2 => "FAIL",
_ => "UNKNOWN",
}
}
fn main() {
let code = 2;
let label = get_status_label(code);
println!("Status: {}", label);
}
// Output:
// Status: FAIL
The returned string is a literal (or potentially a global constant), so it has 'static
lifetime. This pattern is used in libraries like log
, tracing
, and error classification utilities.
'static
as a Bound in Generic Functions
In generic code, you may want to restrict a type to only those that contain no borrowed data — by requiring T: 'static
. This is common in thread spawning, background tasks, or long-lived async operations.
use std::thread;
fn spawn_task<T>(task: T)
where
T: FnOnce() + Send + 'static,
{
thread::spawn(task).join().unwrap();
}
fn main() {
let msg = String::from("Processing complete");
// move forces ownership into closure so it satisfies 'static
spawn_task(move || {
println!("{}", msg);
});
}
// Output:
// Processing complete
Here, the 'static
bound ensures the closure owns all its captured data — necessary because it’s being run in a separate thread, possibly long after the original scope ends.
This pattern shows up in APIs like tokio::spawn
, std::thread::spawn
, and trait objects used for event systems or background workers.
- Use
&'static str
in inputs when accepting string literals or global constants - Return
'static
references only when returning data that truly lives for the entire program - Use
T: 'static
to enforce ownership and safety in threads, async blocks, and trait object lifetimes
'static
in Structs and Traits
When you see 'static
used in structs or trait bounds, it often signals one of two things:
- The type is expected to own all its data, with no borrowed references
- It’s intended for use in long-lived contexts — like background threads, async tasks, or global containers
The 'static
lifetime ensures that any references inside a type are valid for the entire program — or that there are no references at all, because the data is fully owned. It’s both a powerful tool and a common source of confusion, especially when working with trait objects or closures in async
or multi-threaded code.
This section explores how 'static
works in structs and trait bounds, when it’s required, and what it really means for safety and flexibility.
Structs Holding 'static
References
A struct can be declared to hold references with a 'static
lifetime — ensuring it only accepts long-lived or owned data.
struct Command<'a> {
label: &'a str,
}
fn main() {
// a static string literal
static MESSAGE: &str = "Shutdown now";
let cmd = Command { label: MESSAGE };
println!("Command: {}", cmd.label);
}
// Output:
// Command: Shutdown now
In this case, label
holds a 'static
reference — it’s only valid if the input data (like a string literal) lives for the entire program. You can also write Command<'static>
explicitly to enforce this constraint in function signatures or return types.
'static
Trait Bounds (e.g., T: 'static
)
A trait bound like T: 'static
means that T
contains no borrowed references, or that any it does have are themselves 'static
.
struct Container<T: 'static> {
value: T,
}
fn main() {
let name = String::from("Persistent state");
let c = Container { value: name };
println!("{}", c.value);
}
// Output:
// Persistent state
This is useful when you want to ensure a type is fully owned and can be moved freely across threads or stored globally. Any type with internal references must prove they’re also 'static
— which often rules out &str
but allows String
.
Why 'static
Is Often Required in Closures and Async Code
Closures and async tasks are often passed to systems that store or run them long after the current scope ends — such as thread pools or async runtimes. To guarantee safety, Rust enforces that such closures must be 'static
.
use std::thread;
fn launch_job<F>(job: F)
where
F: FnOnce() + Send + 'static,
{
thread::spawn(job).join().unwrap();
}
fn main() {
let log = String::from("Background processing");
launch_job(move || {
println!("{}", log); // moved in, now owned, meets 'static
});
}
// Output:
// Background processing
The 'static
bound ensures the closure owns everything it needs, so nothing can be dropped too early. Without move
, the closure would try to borrow log
, which may not live long enough.
This pattern is also common in tokio::spawn
, crossbeam
, and any framework that stores tasks or handlers internally.
- Use
'static
in structs to hold long-lived references or ensure full ownership - Add
T: 'static
to trait bounds when a type must outlive the current context — often for threading or async use - Rust requires
'static
in closures and async tasks to prevent use-after-free when values are moved across threads or into runtime-managed environments
'static
and Trait Objects
When using trait objects like Box<dyn Trait>
or &dyn Trait
, the compiler needs to know how long the trait object is valid — because trait objects involve dynamic dispatch and may be stored on the heap, moved across scopes, or used in async/threaded contexts.
That’s where the 'static
lifetime often comes in. If you don’t specify a lifetime for a trait object, Rust frequently assumes (or requires) 'static
, especially when the object is:
- Heap-allocated
- Stored
- Spawned into threads
- Used across async boundaries
Let’s explore why 'static
is frequently required in trait object usage and how to distinguish between 'static
and 'a
when creating or passing trait objects.
Why Box<dyn Trait>
Often Needs 'static
When you allocate a trait object on the heap, like Box<dyn Trait>
, you may need to specify 'static
if the trait object might outlive the data it references — or if it’s passed into code that assumes 'static
.
trait Task {
fn run(&self);
}
struct PrintTask;
impl Task for PrintTask {
fn run(&self) {
println!("Running print task...");
}
}
fn spawn(task: Box<dyn Task + Send + 'static>) {
std::thread::spawn(move || {
task.run();
})
.join()
.unwrap();
}
fn main() {
let t = PrintTask;
spawn(Box::new(t));
}
// Output
// Running print task...
Because the task may be executed in another thread, the trait object must be 'static
— meaning it owns all captured data and doesn’t reference anything temporary.
This is typical in thread pools, background schedulers, and async runtimes.
dyn Trait + 'static
vs dyn Trait + 'a
By default, a trait object like &dyn Trait
or Box<dyn Trait>
is ambiguous without a lifetime. You can disambiguate by specifying 'static
(lives forever) or 'a
(tied to a known lifetime).
trait Loggable {
fn log(&self);
}
struct Logger;
impl Loggable for Logger {
fn log(&self) {
println!("Log event captured.");
}
}
fn use_trait_object<'a>(obj: &'a dyn Loggable) {
obj.log();
}
fn use_static_trait_object(obj: Box<dyn Loggable + 'static>) {
obj.log();
}
fn main() {
let logger = Logger;
use_trait_object(&logger); // works with any lifetime
use_static_trait_object(Box::new(logger)); // requires full ownership
}
// Output:
// Log event captured.
// Log event captured.
dyn Trait + 'a
allows references tied to a local lifetime, while dyn Trait + 'static
requires no borrowed data inside and is safe to store or send across contexts.
Heap Allocation and Lifetime Separation
Heap-allocating a trait object (with Box
, Rc
, or Arc
) requires special care with lifetimes. If the inner value includes references, you must declare how long those references live — often meaning 'a
, not 'static
.
trait Describer {
fn desc(&self) -> &str;
}
struct Note<'a> {
msg: &'a str,
}
impl<'a> Describer for Note<'a> {
fn desc(&self) -> &str {
self.msg
}
}
fn print_description<'a>(item: Box<dyn Describer + 'a>) {
println!("{}", item.desc());
}
fn main() {
let text = String::from("This is a borrowed message.");
let note = Note { msg: &text };
let boxed: Box<dyn Describer> = Box::new(note);
print_description(boxed);
}
// Output:
// This is a borrowed message.
You can’t use Box<dyn Describer + 'static>
here because the data inside (text
) is not 'static
. But you can use 'a
, as long as you’re explicit.
- Trait objects like
Box<dyn Trait>
often require'static
because they’re stored or moved across threads - Use
dyn Trait + 'static
when the object owns everything and can live indefinitely - Use
dyn Trait + 'a
when working with borrowed trait objects and you want flexibility - Heap allocation doesn’t require
'static
by default — only when storage or thread safety demands it
Common Pitfalls and Misunderstandings
The 'static
lifetime is one of the most misunderstood features in Rust. While it’s powerful, it’s often misused — either by assuming it means complete safety or by applying it too broadly. Many developers:
- Think
'static
means the value is somehow immortal and always safe - Add
'static
bounds to satisfy an API likethread::spawn
without understanding the implications - Over-constrain types or functions with
'static
, reducing flexibility unnecessarily
Let’s clear up these misconceptions and explains when 'static
is genuinely required — and when it’s not.
Why 'static
Doesn’t Mean “Everything Is Safe Forever”
The 'static
lifetime only guarantees that a value can live for the entire program — it doesn’t automatically make your program safe or leak-free. You can still create bugs, logic errors, or memory bloat with 'static
values.
static CONFIG: &str = "debug=true";
fn show_config() {
println!("Config: {}", CONFIG);
}
fn main() {
show_config();
}
Output:
// Config: debug=true
This is a safe and correct use of a 'static
reference. But if you misuse a 'static
container (e.g. lazy_static!
or once_cell
) and mutate shared state carelessly, 'static
won’t save you from bugs.
Think of 'static
as a promise about data lifetime, not a security blanket.
'static
Required by APIs like thread::spawn
— But Why?
APIs like std::thread::spawn
require 'static
because the new thread may outlive the current function scope. If a closure borrows something locally, it could create a dangling reference when the current scope ends.
use std::thread;
fn main() {
let message = String::from("Task complete");
thread::spawn(move || {
println!("{}", message);
})
.join()
.unwrap();
}
// Output:
// Task complete
The move
keyword forces the closure to own everything it uses, so the compiler can guarantee it’s 'static
(and thus safe to send to another thread).
If you remove move
, the closure may try to borrow message
, which is unsafe because message
will go out of scope before the thread finishes.
Over-Constraining with 'static
When It’s Not Needed
Developers sometimes slap 'static
onto a function or generic parameter just to make the compiler happy — but this can reduce flexibility and usability.
fn print_message<T: std::fmt::Display + 'static>(msg: &T) {
println!("{}", msg);
}
fn main() {
let content = String::from("Hello");
print_message(&content); // works
}
// Output:
// Hello
But the 'static
bound is unnecessary here — msg
is just borrowed for the duration of the call. Removing 'static
improves flexibility:
fn print_message<T: std::fmt::Display>(msg: &T) {
println!("{}", msg);
}
Now, this function works with any lifetime, not just 'static
. It’s still 100% safe — you’ve simply avoided over-constraining it.
'static
means “can live for the entire program,” not “safe no matter what”- Use
'static
when required by thread, async, or global APIs — but know why they require it - Avoid using
'static
unnecessarily — it often reduces flexibility and blocks valid inputs
Lifetimes in Closures
Closures in Rust often capture references from their environment, making lifetimes a central part of how they behave — especially when closures are stored, passed, or returned. While Rust’s inference system often handles lifetimes silently, things can get more complex when closures:
- Outlive the variables they capture
- Are passed to threads, async functions, or long-lived containers
- Are annotated explicitly as function parameters or return types
This section explores how closures interact with lifetimes, how Rust infers lifetime behavior when capturing data, and what you need to know when closures escape their defining scope.
Closure Capture and Inference
Closures in Rust have a unique relationship with lifetimes because they automatically capture values from their surrounding environment. Depending on how a closure uses a captured value, Rust will determine whether it’s captured by shared reference, mutable reference, or by move.
Fortunately, Rust usually infers the correct lifetimes for you — but this behavior is tightly bound to the borrow checker. Understanding when and how Rust inserts these lifetimes behind the scenes helps you avoid common mistakes, especially when closures start escaping the current scope.
Let’s explore how closures capture values, when lifetime annotations are unnecessary, and how the borrow checker interprets closure captures.
How Closures Capture by Reference, Mutable Reference, and Move
By Shared Reference (&T
)
If a closure only reads a variable, it captures it by immutable reference.
fn main() {
let user = String::from("Greg");
let greet = || {
println!("Hello, {}!", user);
};
greet();
greet(); // still allowed — no mutation or move
}
// Output:
// Hello, Greg!
// Hello, Greg!
The closure borrows user
immutably. Rust inserts &user
behind the scenes and tracks the borrow accordingly.
By Mutable Reference (&mut T
)
If the closure mutates a captured variable, it captures it by mutable reference. The variable itself must also be declared mut
.
fn main() {
let mut counter = 0;
let mut inc = || {
counter += 1;
println!("Count: {}", counter);
};
inc();
inc(); // valid — mutable borrow lives through each call
}
// Output:
// Count: 1
// Count: 2
This is equivalent to let mut inc = |&mut counter| {...}
under the hood — the closure gets exclusive access to counter
.
By Move (Own the Value)
If a closure takes ownership, use the move
keyword. This is required when the closure outlives the variable, like in threads or async tasks.
fn main() {
let status = String::from("Running");
let job = move || {
println!("Status: {}", status);
};
job(); // okay — the closure owns `status`
}
// Output:
// Status: Running
move
ensures the closure owns everything it captures — no references. This disconnects the closure’s lifetime from the scope it was defined in, making it valid for 'static
contexts like thread::spawn
.
When Lifetime Annotations Are Unnecessary (Inferred Correctly)
In most common cases, Rust infers lifetimes for closures automatically. When:
- The closure does not escape the function or block
- Captured references are used safely within the closure’s scope
- The closure is called immediately or not stored long-term
…you don’t need any explicit lifetimes.
fn greet_user() {
let name = String::from("Greg");
let say_hi = || {
println!("Hi, {}!", name);
};
say_hi(); // lifetime inferred — name is in scope
}
fn main() {
greet_user();
}
// Output:
// Hi, Greg!
We didn’t write any lifetime annotations, but Rust still tracks lifetimes internally to make sure say_hi
doesn’t outlive name
.
Borrow Checker Behavior with Closure Captures
Rust’s borrow checker tracks closure captures just like any other borrow. If a captured value goes out of scope while still borrowed by a closure, compilation fails.
fn main() {
let closure;
{
let message = String::from("This is temporary");
closure = || {
println!("{}", message); // would borrow `message`
};
}
// closure(); // compile error: `message` does not live long enough
}
Rust prevents closure()
from being called because it would access a dropped value. This ensures safety, even across nested scopes.
- Rust closures automatically capture variables by reference, mutable reference, or move, based on usage
- Lifetime annotations are usually not required unless the closure escapes its original scope
- The borrow checker treats closures like borrowed variables, ensuring they don’t outlive their captures
Closures as Parameters and Lifetime Constraints
Closures are incredibly flexible in Rust — and one of their biggest powers is being passed as function parameters. But when closures borrow values, you’re now juggling not only traits like Fn
and FnMut
, but also lifetimes.
When passing closures that capture references, you may need to annotate:
- How the closure captures its environment (borrowed or owned)
- How long those captured values must remain valid
- Whether the closure is called immediately or stored and reused
Now we’ll explore what it means to pass closures that borrow, how the standard closure traits (Fn
, FnMut
, and FnOnce
) behave with lifetimes, and how to express explicit lifetime constraints when necessary.
Passing Closures That Borrow Data
Closures that borrow from their environment require the borrowed values to stay valid until the closure is used. When passed as parameters, Rust ensures they don’t outlive the references they capture.
fn run<F: Fn()>(f: F) {
f();
}
fn main() {
let msg = String::from("System booting...");
let closure = || {
println!("{}", msg); // borrowed
};
run(closure); // valid — `msg` lives long enough
}
// Output:
// System booting...
This works because msg
lives for the entire main()
scope, and closure
doesn’t outlive it. Rust infers the borrow and ensures it’s safe to call f()
.
If run()
had stored the closure instead of calling it immediately, you’d need explicit lifetime annotations.
Using Fn
, FnMut
, and FnOnce
Traits with References
These three closure traits dictate how the closure uses captured variables:
Fn
: borrows immutably (&T
)FnMut
: borrows mutably (&mut T
)FnOnce
: takes ownership (moves)
Each has different lifetime implications.
fn call_twice<F>(mut f: F)
where
F: FnMut(),
{
f();
f();
}
fn main() {
let mut count = 0;
let mut counter = || {
count += 1;
println!("Count: {}", count);
};
call_twice(&mut counter); // requires FnMut
}
// Output:
// Count: 1
// Count: 2
The closure captures count
by mutable reference, so it implements FnMut
. The lifetime of the borrow is inferred to cover both calls to f()
.
You can switch to FnOnce
by capturing owned data (e.g., String
) and using move
.
Explicit Lifetime Bounds When Closures Are Stored or Reused
When a closure borrows data and you store it for later use, Rust needs to know how long the borrowed data will live. This is where lifetime annotations become mandatory.
fn store_callback<'a, F>(callback: F) -> Box<dyn Fn() + 'a>
where
F: Fn() + 'a,
{
Box::new(callback)
}
fn main() {
let greeting = String::from("Welcome!");
let cb = store_callback(|| {
println!("{}", greeting); // borrow
});
cb(); // valid — `greeting` still lives
}
// Output:
// Welcome!
The closure borrows greeting
, so we must tie the trait object (dyn Fn()
) to the same 'a
lifetime. Without 'a
, the compiler would assume 'static
, which wouldn’t be valid here.
This pattern is key in GUI frameworks, event handlers, or any system where closures are stored and executed later.
- Passing closures that borrow values works as long as the borrowed data lives long enough
Fn
,FnMut
, andFnOnce
influence how a closure captures — and therefore how long it can be safely used- When storing closures that borrow, lifetime annotations like
'a
are required to make the reference lifetimes explicit
Closures Returning References
Closures that return references are subject to Rust’s strict borrowing rules. When a closure returns a reference — especially one that points to captured data — you often need lifetime annotations to tell the compiler how long the returned reference will be valid.
In some simple cases, Rust can infer the correct lifetime. But when the closure is used generically, stored, or passed around, you must explicitly declare which lifetimes are related — particularly between captured data and the returned value.
This subsection explores when returning references from closures requires lifetimes, how to express them clearly, and where inference reaches its limits.
When Closure Return Types Require Lifetime Annotations
Rust requires explicit lifetime annotations when a closure returns a reference and its lifetime relationship is ambiguous — such as when storing the closure or using it generically.
fn make_getter<'a>(input: &'a str) -> impl Fn() -> &'a str {
move || input
}
fn main() {
let msg = String::from("Status: OK");
let getter = make_getter(&msg);
println!("{}", getter());
}
// Output:
// Status: OK
The closure captures input
by reference and returns it. Because it escapes its defining scope (returned from a function), we must declare that the returned reference will live at least as long as 'a
.
Without the <'a>
annotation on the function, this would not compile.
Tying Return Lifetimes to Captured Values
You may want a closure to return a reference to something it captures — and tie the output lifetime to the lifetime of the input or captured value. Here’s a case where lifetime inference does work:
fn main() {
let data = String::from("Hello, Rustacean!");
let get_first_word = || {
let pos = data.find(' ').unwrap_or(data.len());
&data[..pos]
};
println!("First word: {}", get_first_word());
}
// Output:
// First word: Hello,
Because get_first_word
doesn’t escape the function and is only used while data
is valid, Rust can infer that the returned reference is valid — no lifetime annotations needed.
But if you try to return this closure or store it, you’ll need to specify the output lifetime explicitly.
Limitations with Inferred vs Explicit Lifetime Relationships
The moment you try to use such closures as trait objects or function return values, lifetime inference is no longer enough — Rust needs help.
fn make_trimmer<'a>(s: &'a str) -> Box<dyn Fn() -> &'a str + 'a> {
Box::new(move || s.trim())
}
fn main() {
let msg = String::from(" system ready ");
let trim_fn = make_trimmer(&msg);
println!("Trimmed: '{}'", trim_fn());
}
// Output:
// Trimmed: 'system ready'
The returned reference from the closure is tied to the lifetime 'a
of the input &'a str
, so we must annotate it. Without 'a
, Rust assumes 'static
— and the borrow checker would reject it.
- When a closure returns a reference, Rust needs to know how long that reference will remain valid
- If the closure doesn’t escape the current scope, inference usually works
- If the closure is stored, returned, or used generically, explicit lifetime annotations are required to describe the relationship between the closure and the data it returns
Long-Lived Closures: 'static
and Lifetime Boundaries
Closures passed to long-lived tasks — such as threads, async runtimes, or event loops — must live long enough to remain valid for their execution. That’s why APIs like thread::spawn
or tokio::spawn
require closures that are 'static
— meaning the closure captures either:
- No references at all, or
- Only references to
'static
data, or - Takes ownership of captured values so the data lives inside the closure
This subsection explores why these APIs enforce 'static
, how to meet that requirement using move
, and how to avoid adding 'static
unnecessarily where it limits flexibility.
Why Closures Passed to thread::spawn
or tokio::spawn
Require 'static
These APIs require 'static
closures because the closure is spawned independently and may run after the original scope ends. Borrowing local data would be unsafe.
use std::thread;
fn main() {
let msg = String::from("Hello from thread");
let handle = thread::spawn(move || {
println!("{}", msg); // moved, not borrowed
});
handle.join().unwrap();
}
// Output:
// Hello from thread
This works because the closure is marked move
, which takes ownership of msg
. The closure becomes 'static
because it no longer relies on anything outside its body.
Without move
, the compiler would prevent the closure from borrowing local variables, since the thread may outlive them.
Moving Captured Values to Satisfy 'static
The most common way to satisfy 'static
is using move
, which moves captured data into the closure, making it independent of the outer scope.
fn spawn_with_value() {
let data = String::from("Detached job");
std::thread::spawn(move || {
println!("Running with: {}", data);
})
.join()
.unwrap();
}
fn main() {
spawn_with_value();
}
// Output:
// Running with: Detached job
Once data
is moved, the closure no longer borrows anything — so it qualifies as 'static
. This lets it be sent across threads safely.
This technique is also used in tokio::spawn
, rayon
, and event queues.
Avoiding Unnecessary 'static
by Being More Precise
Sometimes developers add 'static
bounds to function parameters just to satisfy the compiler — but this can limit reusability and exclude perfectly valid inputs.
fn run_job<F>(job: F)
where
F: Fn() + 'static,
{
std::thread::spawn(job).join().unwrap();
}
❌ This restricts callers to pass only 'static
closures. But if job
is executed immediately, 'static
isn’t needed.
A better alternative when you don’t need to store or spawn the closure is:
fn run_inline<F: Fn()>(f: F) {
f();
}
fn main() {
let note = String::from("Running now");
run_inline(|| {
println!("{}", note); // borrowed — and that’s fine
});
}
// Output:
// Running now
Since f
runs immediately, the closure’s lifetime is short and local — no need for 'static
.
Use 'static
only when the closure is passed to something that will outlive the current stack frame.
- Closures passed to
thread::spawn
ortokio::spawn
must be'static
to avoid dangling references - Use
move
to own captured values and make the closure'static
- Don’t overuse
'static
— only require it when the closure will be stored, spawned, or run later
Common Closure Lifetime Pitfalls
Closures offer concise, expressive power in Rust — but they also expose common pitfalls related to borrowing, ownership, and lifetime inference. These pitfalls often confuse developers, especially when closures interact with the borrow checker in ways that aren’t immediately obvious.
Let’s look at common lifetime mistakes in closure usage and explain what the compiler is trying to protect you from — and how to resolve those issues cleanly.
Attempting to Borrow Data from Outside a Closure After It’s Moved
One of the most common lifetime mistakes is trying to use a value after it’s been moved into a closure.
fn main() {
let data = String::from("Important");
let print_data = move || {
println!("Inside closure: {}", data);
};
// println!("Outside: {}", data); // ❌ compile error: value moved into closure
print_data();
}
// Output:
// Inside closure: Important
move
takes ownership of data
. Attempting to use it after the closure is created causes a “value used after move” error. This isn’t a lifetime issue per se — but it becomes one when misunderstood in longer lifetimes or 'static
contexts.
Fix: Clone the value if you need it both inside and outside.
Confusing Closure Inference with Function Lifetime Elision
Developers often expect closure inference to behave like function lifetime elision — but closure inference is stricter in some cases.
fn main() {
let msg = String::from("hello");
let get_ref = || -> &str {
&msg
};
// ❌ error: missing lifetime specifier
println!("{}", get_ref());
}
// Output:
// hello
The compiler needs to tie the lifetime of the return value to msg
, but closures don’t use lifetime elision rules the same way as free functions.
Fix: Use a named lifetime when necessary (if storing or returning the closure), or don’t return borrowed data from closures that escape scope.
Errors Like “Borrow May Not Live Long Enough” — What It Means
This error occurs when a captured borrow might outlive the value it refers to — often due to storing closures or returning them.
fn make_closure<'a>() -> impl Fn() + 'a {
let local = String::from("temporary");
// ❌ borrow may not live long enough
move || println!("{}", local)
}
fn main() {
let closure = make_closure(); // local is dropped here
closure(); // invalid
}
// Output:
// temporary
The closure tries to capture local
, but local
is dropped at the end of make_closure()
. The compiler catches this and prevents a dangling reference.
Fix: Either extend the lifetime of the captured value, or move it in from outside:
fn make_closure<'a>(data: String) -> impl Fn() + 'a {
move || println!("{}", data)
}
fn main() {
let text = String::from("safe now");
let closure = make_closure(text);
closure(); // OK
}
// Output:
// safe now
- Moving data into a closure transfers ownership — don’t reuse the value after
- Lifetime inference in closures is not as flexible as in functions — especially for return types
- “Borrow may not live long enough” means the closure might outlive the captured borrow — fix it by moving or restructuring the closure’s scope
As we’ve seen, lifetimes are a powerful part of Rust’s type system that allow it to guarantee safety without runtime overhead. While the syntax can feel overwhelming at first, lifetimes give us precise control over how data is accessed and shared—enabling us to write robust, high-performance code.
With this knowledge, you’re better equipped to understand lifetime errors, write safe APIs, and confidently work with references in increasingly complex scenarios.
Thanks very much for including ByteMagma in your journey toward Rust mastery!
Leave a Reply